The traces of the universe’s earliest stars may have been found after observations of a distant quasar uncovered evidence of a first-generation star that died in a ‘super-supernova’ explosion. But how do you observe things that exploded billions of years ago? The University of Notre Dame’s Professor Timothy C Beers explains.
DOI.ORG/10.58214/UND0103

Profile: Timothy C Beers
Timothy C Beers is Grace-Rupley Professor of Physics at the University of Notre Dame. His key interest is the origin and evolution of the elements in the universe, and the assembly of large spiral galaxies such as the Milky Way, a field now referred to as Galactic Archaeology. For decades, Beers has designed and executed large-scale surveys of stars in the Milky Way, sifting through millions of individual stars in order to find those objects that have recorded the chemical history of the universe in their atmospheres.
Fig. 1. The Milky Way (above) has something like 200 to 400 billion stars
Our galaxy, the Milky Way, contains between 200 and 400 billion stars, the vast majority of which are far too faint to be visible in this image. Sorting out WHICH of these stars contain the precious chemical information that astronomers seek to learn about the early chemical evolution of the universe is a daunting challenge, but one which is being undertaken with new-generation photometric and spectroscopic surveys. © Bigstock Images @ aslysun.
Astronomers believe they may have discovered the ancient chemical remains of one of the first stars to light up the universe. Using an innovative analysis of a distant quasar observed by the 8.1-meter Gemini North telescope on Hawai‘i, operated by the USA’s National Science Foundation’s NOIRLab, the scientists found an unusual ratio of elements that, they argued, could only come from the debris produced by the all-consuming explosion of a 300-solar-mass first- generation star.
The research (https://ui.adsabs.harvard.edu/link_gateway/2022ApJ…937…61Y/PUB_PDF) is the result of decades of work into finding the earliest-ever stars to inhabit the universe. And it is no mean feat. Aether talked to Professor Timothy C Beers, Grace-Rupley Professor of Physics at the University of Notre Dame who worked on the research with his long-term collaborator, co-author of the recent paper, Professor Yuzuru Yoshii of the University of Tokyo.
Beers outlined the science behind the announcement and his journey as an astronomer which has seen myriad changes in approaches and methods of data gathering and observation as the world has gone digital and ever faster and more powerful instruments have become available to the field of astronomy.

Fig. 2 PISN pre-explosion: Interior of a massive star about to explode as a PISN. At the high temperatures reached in the core of such stars, pairs of electrons and positrons are created, which reduces the radiation pressure in the centre needed to push back against gravity. A partial core collapse follows, triggering the explosive nucleosynthesis of elements (such as magnesium and iron) that are used to identify a PISN. Credit: NASA/ CXC. Public domain.
Aether: Can you give a little background into the research that’s gone before you in this area?
TB: Astronomers, including Professor Yoshii and myself, have been fascinated for decades to see if we could find evidence for the presence of the very first stars born in the universe.
We know the process had to start at some point, and the evidence up to now has pointed towards stars of that very first generation being quite massive, many times more massive than the sun. Simulations, which have improved over the last few decades, predict a large range of masses; anywhere from ten to a thousand times the mass of the sun – that’s a pretty big range.
What has occurred more recently is that the bulk of evidence gathered from a variety of directions indicates that the mass range for most of the very first stars is probably closer to 150 to 300 times the mass of the sun.
In order to advance our understanding, we have to find and verify that evidence. In this mass range stars live a very short life, a few million years at the most, because they rapidly burn their available fuel. When they die, their explosion has a different character than what we find happening with lower mass stars.
The explosion, a ‘pair-instability supernova’ (PISN), is a challenging conceptual image. A PISN is a type of supernova predicted to occur when the production of pairs of free electrons and positrons (a particle of anti-matter with the mass of an electron but a positive charge) from the transformation of energetic gamma rays that are present at the extreme temperature of the core.
This effectively robs the star of the radiation pressure that supports its core, pushing back against gravitational collapse. This leads to a partial core collapse, which in turn causes greatly accelerated burning in a runaway thermonuclear explosion, resulting in the star being blown completely apart, with no remnant. (See Fig. 2)
The thing that matters for us is that this particular kind of explosion yields a characteristic elemental-abundance pattern. The nucleosynthesis that occurs during that explosion is very different than what we see in lower mass stars, owing to the extreme energetic conditions for a PISN.
For decades, astronomers have been looking to find evidence of that particular elemental-abundance pattern, and that has proved to be a challenge. Over the last ten years or so there have been claims made that such explosions have been seen, typically at high redshift, of stars that look to have a different light curve than other known classes of supernovae.
A light curve describes how the luminosity, or the total energy, of an explosion drops off with time. Most supernovae we study today are very similar, but PISNe were predicted to have a different looking light curve, and a number of claims have been made for their detection.
The problem is that, with such a claim, it’s a one-off. In other words, you have an explosion and you track it for a few days to a few weeks, and then it’s done. It is never going to explode again. You only have one shot.
For many researchers that is not satisfactory; the modelling involved is uncertain and the observations are at best few in number. So, while there is a notion that these explosions may have been seen, it’s never really been definitive.
The reason why Prof Yoshii and I started working together almost 30 years ago was to look for chemically primitive, long-lived, lower-mass stars that we can identify today in the Milky Way, and try to find the characteristic elemental-abundance pattern associated with the PISNe explosions that polluted the gas from which they formed. The idea was that we could study something that is now long gone; the exploded object, by looking at what had left behind.
I like to think of them as fossils of the early chemistry of the universe, and this is where it gets really interesting; the idea is that if we find stars that are very old and try to find the oldest fossils that we can, we expect some of those to have inherited this characteristic signature from the first stars.
But the very oldest stars are difficult to find. They are quite rare. So, for more than 30 years, I and my colleagues have carried out extensive surveys in the Milky Way to discover these very ancient stars.
Aether: How do you know a star is ancient?
TB: Today we have techniques that are improving direct age estimates, but that’s not how we do it.
We look for stars that have very low abundances of metals (the term astronomers use to refer to all elements heavier than hydrogen and helium), the notion being that if you go back to the very beginning, there were very few stars available to have polluted the gas from which a star formed. Therefore, as you go to lower and lower abundances of metals, you are simultaneously looking at more chemically primitive times. (See Figs. 3 and 4)
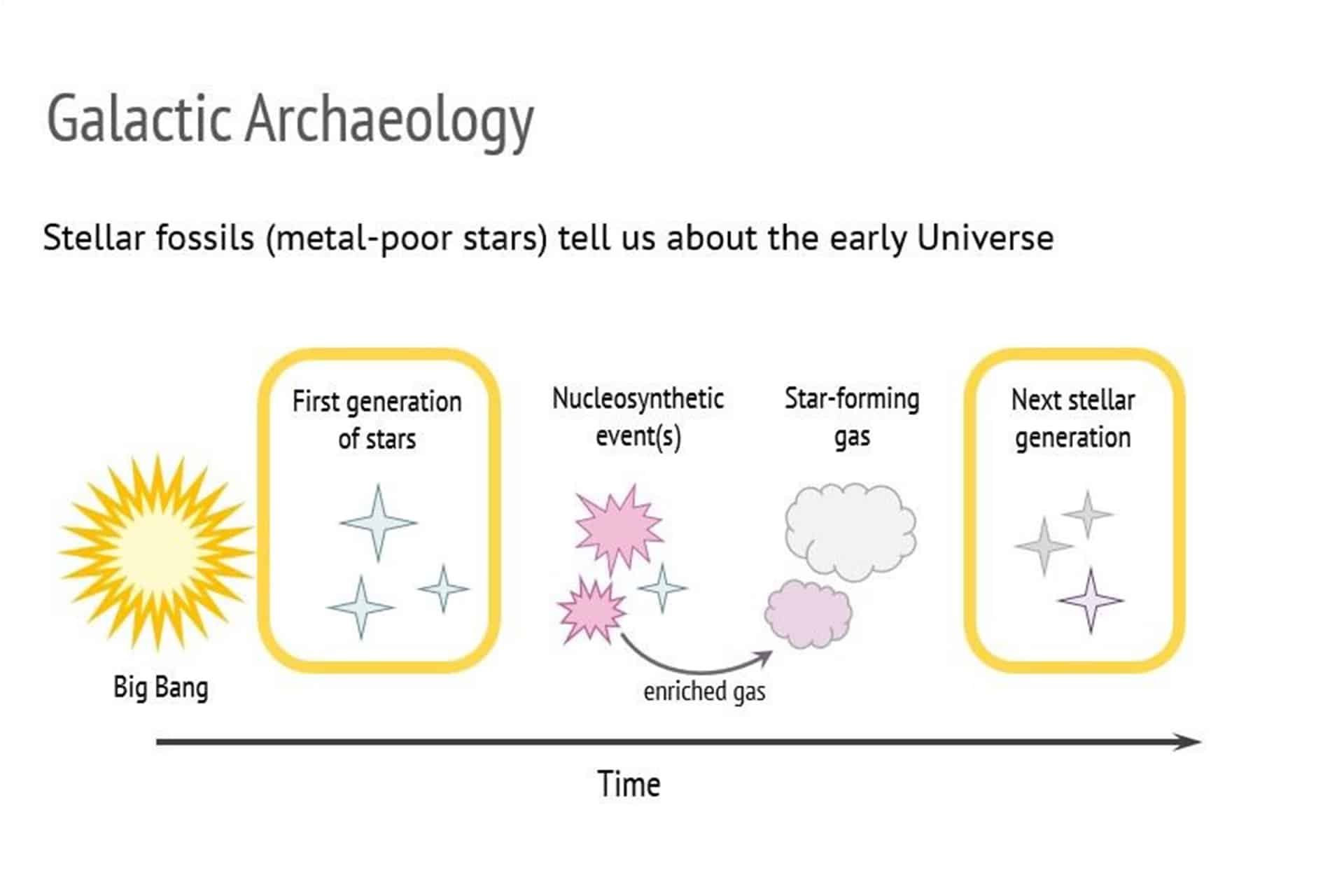
Fig. 3. Chemical history from stars
Chemical history from stars – The pathway to origin of elements in the Periodic Table. The Big Bang creates hydrogen and helium, but all heavier species are created by subsequent generations of stars. The very first stars were likely quite massive, including those thought to explode as PISNe. Enrichment of the remaining gas enables lower mass stars to form, some of which are expected to have recorded the characteristic abundance patterns predicted from PISNe. © Erika Holmbeck.

Fig. 4. Stellar metallicity as a tracer of chemical evolution
Multiple supernovae explosions of early generations of stars enrich the gas from which later generations of stars form, and seen in the right panel. In the left panel, a representation is shown of the high-resolution spectra of stars in the first stages of this process. At first, there are NO elements seen in the spectrum, then subsequent explosions create elements that can have detectable lines. Examples are shown in the middle two spectra. After about nine billion years, enrichment has resulted in numerous and much stronger lines, like the spectrum of the sun in the bottom panel. © Norbert Christlieb.
Astronomers have now done that. We have identified and studied a sufficient number of these ancient stars and have a basic understanding of the chemical-abundance patterns that they reveal. Up to now, however, none of those has definitively matched the predicted pattern for PISNe.
That would count as a failure, but we have come to the realisation that we may have been looking in the wrong place all along to find the chemical traces of PISNe. The reason is that predictions of the explosions of PISNe typically produce large amounts of iron.
Iron is an element astronomers use to establish if a star is truly ancient. If we are looking for stars that have very low iron abundances to assign them to this ancient category, those stars may not be what we expect to find from a PISNe explosion. There is a tension here. By trying to find evidence for ancient stars using low iron abundance as a probe, we may be looking at progenitor objects that do not include PISNe.
We can find many stars with high iron abundance – that is not a problem. The difficulty is that iron can be made in many different ways, only one of which might be from a PISN. There are millions of iron-rich stars we could study that may have nothing to do with PISNe, which means we now have the opposite problem – too many objects to sort through.
Our current discovery is based on a spectrum of gas associated with a high-redshift quasar, which is not a star, but the centre of an active galaxy where there is a supermassive black hole which gas and stars are falling into, generating tremendous amounts of energy.
Astronomers have been using these high-redshift quasars for a long time in order to understand galaxy formation and cosmology, amongst other things. A spectrum taken of one particular quasar Prof Yoshii and the other authors on the paper argue matches the predicted elemental-abundance signature of a PISN, in particular traced by the contrast of the abundance of iron and magnesium.
The quasar did not make the elements; it just illuminates the gas that has already been polluted by the explosion of a PISN. As expected, the iron abundance is high, but what is interesting is that the magnesium abundance is low. The contrast of high iron and low magnesium is one of the predictions of the nucleosynthesis from stars in the 150-300 solar mass range.
Perhaps we can use this discovery to teach us how to look for evidence of PISNe pollution of stars in the Milky Way. If magnesium is low relative to iron in a given star (by factors of ten or more compared to the solar ratio), it is likely to have formed from gas that inherited the nucleosynthesis products of a PISN. From this point of view, we should actually be searching for stars that have this characteristic pattern, rather than simply stars thought to be the most chemically primitive.
We are just beginning to use this probe, and I believe this is the direction the research will go in the future. Of course, there will also be more observations of high-redshift quasars with the James Webb Space Telescope and large ground-based telescopes seeking to verify that the signature we have discovered is common for such objects.
Aether: How are your recent discoveries guiding you into what to look for next?
TB: How do we find stars with relatively low magnesium abundance? Traditionally, the way we obtain the abundance of an element is by dispersing the light into a spectrum. We then analyse that spectrum, compare it with models, and use that comparison to infer how much of a given set of elements is present – a technique referred to as `spectral elemental- abundance analysis’.
This kind of work has been going on for decades. It might take anywhere between 20 minutes to a few hours with a large telescope to obtain a sufficiently high signal-to-noise spectrum of a star. One star at a time. The problem is that the Milky Way has something like 200 to 400 billion stars, so you are never going to get through them all, or even a significant fraction of them. (See Fig. 4)
That is why the hope was that the very rarest stars, the chemically primitive fossils, which are few in number, would exhibit the abundance signature of a PSIN. So far, this hasn’t proven to be true. If there was another way to obtain elemental-abundance information more quickly, and to make a legitimate attempt to categorise hundreds of millions of stars, maybe we can start finding these very rare, exceptional cases such as stars with relatively low magnesium.
To that end, astronomers have developed a technique called ‘multi-fibre spectroscopy’. The first large-scale example of that was the Sloan Digital Sky Survey. In this approach 650 to 1,000 fibres in the focal plane of a wide-field telescope, each fed simultaneously to one or more spectrographs, are used to gather numerous spectra simultaneously. In the time it would take me to get one spectrum by the traditional approach, Sloan could obtain up to a thousand.
In the 20+ years since Sloan started, astronomers in the modern era can now obtain up to 5,000 spectra at a time. The numbers are becoming huge. A million spectra is a big number, let alone a hundred million, but this still represents much less than 1% of the total number of stars in the Milky Way. Thus, astronomers have started to wonder whether there was an even better route than spectroscopy alone.
That better way is by just taking a picture; in other words, measuring the light output of a star in a number of precisely defined filters – a technique known as `photometry’. Instead of dispersing the light from individual stars you simply point a telescope in a certain direction and take a picture in multiple filters.
Photometry is similar to taking a picture with your smartphone, but when we take an astronomical picture, we have much better control over our filters. The innovation was to construct narrow-band filters that only pass a very small amount of the light, and centring those filters on the absorption lines that correspond to individual elements. (See Fig. 5)
This approach has now developed into a technique that I believe will solve, or at least will take us far down the road, towards resolving the problem of how to obtain elemental-abundance information for hundreds of millions of stars.
From my perspective, the next step in this is really twofold: one, there’s certainly going to be more high-resolution spectra of distant quasars; and two, searching through literally hundreds of millions of stars in the Milky Way galaxy to find those stars that are very low in magnesium compared to iron.
Once we find such stars, which could number very few, from the tens to hundreds of stars, they can be studied at high spectral resolution with large telescopes, because we know exactly which ones to point at.
The surveys I am most closely involved with are called J-PLUS (Javalambre Local Universe Survey), which uses a relatively small, automated telescope in Spain, and S-PLUS (Southern Local Universe Survey), which employs identical filters on an identical telescope located in Chile.
Over the past several years, I have been working with colleagues to calibrate and validate this technique, and, in the next few months, we’ll be publishing the results of those calibrations. So far, they all look good.
We think this is a better way; it is faster and almost as accurate as taking a spectrum. It provides a straightforward path for finding stellar-abundance evidence for PISNe in the early universe.

Fig. 5. Narrow- and Medium-band filters
Narrow- and medium-band filters used by J-PLUS and S-PLUS to rapidly obtain information about the abundances for a limited number of elements (importantly, Mg and Fe) for each star imaged through the filters. The narrow-band filters are located where detectable lines in a spectrum are found, while the medium-band filters help with the determination of the temperature and surface gravity of a star. Thousands to tens of thousands of stars can be imaged at once. © SPLUS (https://www.splus.iag,usp.br/).
Aether: You have spent a significant amount of your working life looking up at the night sky – is it the case that you are hoping to get lucky and see something or are you following clues?
TB: The term is `survey’ and you hear it all the time. It means an organised attempt to find what you’re looking for. What others and I have done is develop processes optimised for looking for the ancient chemically primitive stars, the fossils.
We started taking pictures of the stars, but stars are points of light, so we didn’t get much from broad-band filter pictures. Then we developed a technique using very low-resolution spectra, which allowed us to get many, perhaps 10,000 at a time, then go through those individual spectra, initially using visual inspection, later computer-scans. We would pick out the ones that looked like they might be interesting, that is they had some evidence of being ancient, meaning they were metal-poor with low abundances of iron and other elements.
With random spectra you would never get there. This was a process, a bit like using a sieve, and in the early days, if we got a 20% success rate, we were thrilled.
Today, with our refined techniques, we can achieve above a 50% success rate, maybe 70 or 80%, which is outstanding. That’s the way we direct our searches. But even that approach, clever though it might be, doesn’t get you hundreds of millions of stars; we’d like to study the entirety of the Milky Way, but the numbers are overwhelming.
Going forward, astronomers have come to realise that we have to change our techniques. We will hear about lots of surveys in the next five years or so, which are still taking large amounts of spectra. This is good, and they will be useful, but my personal guess is that we are going to learn more from the photometric surveys, the ones which are taking pictures with precise filters, than we will from the spectroscopy alone.
Spectroscopy and photometry are complementary techniques. If it is raw numbers that you want, then you may have to go through hundreds of millions of stars to find a handful of the most interesting ones, which is what I think it will take for finding definitive evidence for PISNe in the early universe. For this application, you can’t beat the photometric approach. It is so fast, does not require huge telescopes, and our detectors are so good these days that we can record almost every photon that falls on a detector.
Aether: What is your personal end goal in this field and how do you think your work is going to prove useful in the future?
TB: What has happened over the years, and to which I have made a contribution, is finding stars with a variety of elemental-abundance patterns, and learning how to interpret those patterns to tell us where those chemical abundances came from.
I look at the Periodic Table in a very different way from most people. Today we actually have a pretty good narrative as to the astrophysical origins for almost all of those elements. They weren’t all created in the same way; there were different nucleosynthesis processes, operating in different sites, and different chemical histories that led to the different kinds of elements.
Over the last few decades, we have been piecing together what I believe to be a coherent first draft of where the Periodic Table came from. To me, that’s already an amazing accomplishment.
Where we are going next is to take that very rough outline of the origin of all the elements and fill in the details. It is like chemistry in reverse. We want to understand the history of how the Periodic Table got there. To me, it’s been fascinating just to get to the point now where we can tell this plausible story of the origin of the elements, one that will be greatly refined in the near future.
My laboratory is the universe, and I’m studying things that exploded in that laboratory 12 to 13.5 billion years ago, based on fossil stars that have recorded evidence of their progenitors over that enormous expanse of time.

Timothy C Beers
Grace-Rupley Professor of Physics
Department of Physics and Astronomy
University of Notre Dame
https://physics.nd.edu/
Fig. 6.
The step-by-step story of how astronomers may have discovered the ancient chemical remains of the first stars to light up the Universe. Using an innovative analysis of a distant quasar observed by the 8.1-metre Gemini North telescope on Hawai‘i, operated by NSF’s NOIRLab, the scientists found an unusual ratio of elements that, they argue, could only come from the debris produced by the all-consuming explosion of a 300-solar-mass first-generation star.
© NOIRlab/ NSF/ AURA/ J de Silva/ Spaceengine.
(CC BY)